Geiger Counters
Background
Geiger counters, also referred to a Geiger-Müeller detectors, are survey detectors used to determine the presence of radiation and abundance of radiation. Geiger counters produce readings of count rate or integrated number of counts. Readings can be converted to units of exposure (Roentgen) in the presence of a photon source.
Uses
- Detect presence and relative abundance of radiation
- Accuracy typically +/-20%, +/-10% achievable
- Radiation surveys
- Leak tests
Advantages
- Highly sensitive even to very low levels of radiation
- Ideally suited for detection of leakage radiation or contamination
- Collected charge per count is high which does not require complex electronics to measure
- Inexpensive
Disadvantages
- Dead time accumulation
- Dead time ~10-100ms
- Limits accurate count rates to a few hundred counts per second
- Can read near-zero within a clinical beam
- Unable to determine energy
- Measurements of exposure are inherently inaccurate unless calibrated to a known spectrum
Key point: Geiger counters are not suitable for measurement of linac produced fields. This is because the instantaneous fluence of a such pulsed fields causes excessive dead time accumulation.
Theory of Operation: Geiger Discharge
Geiger discharge is an amplification process which allows Geiger counters to be highly sensitive to the presence of ionizing radiation. A Geiger discharge is started when an electron is ejected from an atom by ionizing radiation and begins a Townsend avalanche. The electron is accelerator by a high electric field gradient toward an anode, gaining sufficient energy along its path to produce more ionizations. Electrons liberated in this way are also accelerated toward the anode causing an avalanche effect. In addition to electron induced ionizations, some ionized atoms return to their ground state via emission of photon. These photons go on to ionize additional atoms and additional Townsend avalanches in a net process known as the Geiger discharge. Geiger discharges commonly result in 109-1010 electrons reaching the anode for a single initial ionization event!
The entire Geiger discharge process takes only a few microseconds. While the electrons are accelerated rapidly toward the anode, their positively charged ions remain effectively stationary due to their much greater mass. During the discharge, these positively charged ions accumulate in the chamber’s fill gas and reduce the voltage difference between themselves and the anode. This reduced voltage eventually leads to the termination of the Geiger discharge as liberated electrons no longer gain sufficient energy to induce further ionization.
The positively charged ions eventually drift to the cathode where they collect an electron and return to their ground state. This process requires some time, 10+ of milliseconds, during which the chamber experiences dead time (internal link).
Key point: Townsend avalanche refers only to the cascading ionizations caused in electron induced ionizations. A Geiger discharge includes many Townsend avalanches induced by photoelectric absorption of photons emitted during atomic relaxation.
Design
Geiger counters consist of a Geiger-Müller (GM) tube and an electronic counting/processing system. Geiger-Müller tubes are large volume ionization chamber operated in the Geiger-Müeller voltage region (~1000V). Geiger-Müller tubes are filled with an inert noble gas, usually helium or argon, to provide chemical stability during repeated ionizations. An additional gas, known as a quench gas, is sometimes also incorporated to reduce false signals generated as the ionized fill gas returns to ground state after a discharge.
GM tubes are usually sealed with a mica entrance window and operated at less than atmospheric pressure. Operating at reduced pressure increases the average accelerating time for liberated electrons by reducing the number of low energy, non-ionizing, collisions and thereby maximizing amplification.
Quench Gases and External Quenching
Following a Geiger discharge, the positively charged fill gas ions return to their ground state by collecting an electron from the cathode. During this process the atom releases energy equal to the difference between the vacant shell binding energy and the ground state. If this released energy is sufficient to induce an ionization, additional Geiger discharges can be produced which causes false readings. Two methods exist to mitigate this effect; external quenching and the incorporation of quench gasses.
External Quenching
External quenching reduces the voltage to the GM tube for a fixed period following a reading, thereby preventing false readings. This method can be achieved very simply by choosing a resistor/capacitor combination of the readout electronic such that the time constant is large. The main disadvantage of external quenching is that it greatly increased dead time, making the unit only useful for very low count rates.
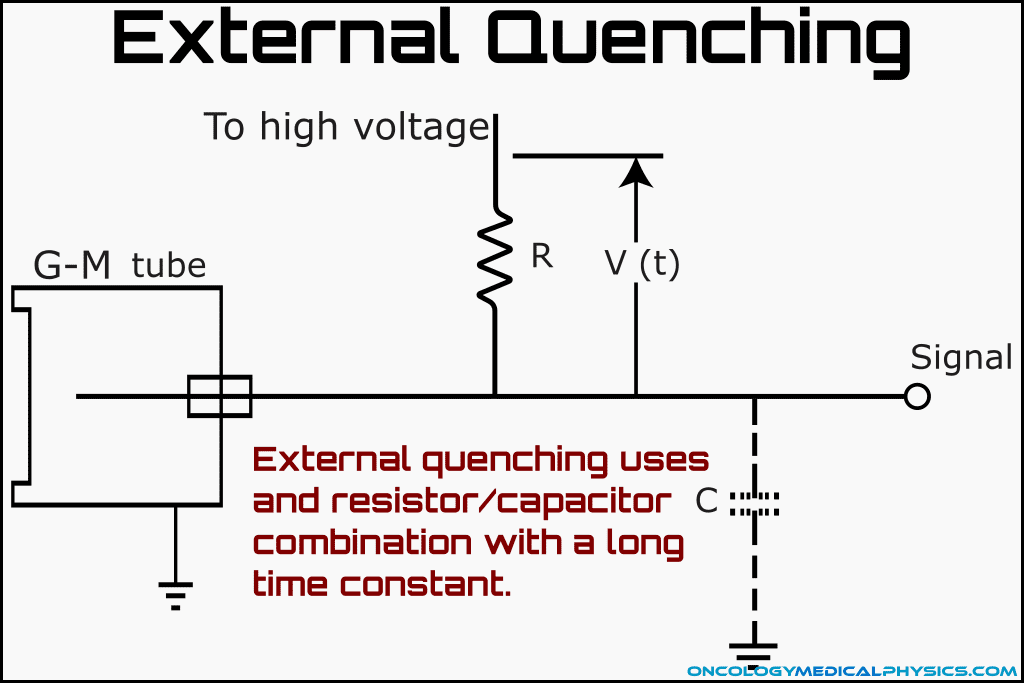
Quench Gas
Quench gasses are complex molecules with lower ionization potential than the noble fill gas. Because of its lower ionization potential, the quench gas is able to provide electrons to the noble gas which allows the noble gas to return to ground state. The complex fill gas then travels to the cathode instead of the noble gas where it is more likely to reduce energy via disassociation than via electron emission. Quench gasses are usually organic molecules such as ethyl alcohol or ethyl formate and incorporated at a rate of about 5-10% of the total fill gas.
Incorporation of a quench gas is much more common than use of external quenching because of its ability to reduce dead time. The main disadvantage of quench gas use is that the disassociation of quench gasses is a destructive process, limiting the life of the gas as it is consumed. This disadvantage can be mitigated through the use of halogens (chlorine or bromine) which are able to reassociate following disassociation.
Key point: Although the term quench gas is used for both proportional counters and Geiger counters, it has a distinct meaning in each context.
- Proportional counter quench gas exists to absorb UV photons, thereby preventing them from creating additional Townsend avalanches and initiating a Geiger discharge.
- Geiger counter quench gases exist to supply electrons to the fill gas and disassociate, thereby preventing additional Geiger discharges as the fill gas returns to ground state.
Radiation Specific Designs
High Energy Photons (X-ray and Gamma Rays) Designs
Most high energy photons are detected via interaction with the detector wall rather than the gas directly because of the gasses low attenuation coefficient. Counting efficiency is dependent upon the probability that the incident photon interacts with the chamber wall. The probability that the induced electron reaches the fill gas. Increased wall thickness increases probability of interaction but decreases probability of an electron reaching the fill gas and the net effect essentially cancels dependence on wall thickness beyond the electrons expected path length. High Z materials should be used for the chamber wall as they maximize the probability of interaction without greatly impacting path length. Bismuth (Z=83) is a common choice. With such designs, counting efficiencies of around 2% can be achieved.
Low energy photons interact directly with the fill gas in large numbers. This can cause overresponse if broad spectrum counting is desired. Designs intended to maximize response to low energy photons use high atomic number fill gasses and high chamber pressures to maximize interaction probability. Such chambers are capable of nearly 100% counting efficiency for photon energies below 10keV.
Charged Particles Designs
Counting efficiency for charged particles is limited by the probability that an incident charged particle will pass through the window. Therefore use of a thin window is greatly desired especially for measurement of high LET particles such as alpha particles. High energy electron count efficiency can near 100% while higher LET particles and lower energy particles experience significant reductions in efficiency.
Neutrons Designs
Thermal neutrons can be detected with a specially designed GM tube using a fill gas with a high neutron capture cross section such as 3He. Fast neutrons can also be detected, to some degree, due to interactions in the GM tube wall. Windows of neutron specific GM tubes are thick to reduce the impact of charged particle and low energy photon contamination. Such designs are uncommon however as the GM tube’s all-or-nothing response makes discriminating neutrons from incident photons impossible. Such tube are better operated at proportional counter voltages to allow photon discrimination.
Navigation
Not a Premium Member?
Sign up today to get access to hundreds of ABR style practice questions.